Impulse Noise Produced by Weapons: Implications for Hearing Conservation
NOTE: THIS ARTICLE ORIGINALLY APPEARED IN SPECTRUM (Summer 2014)- the official organ of the NHCA. We thank the NHCA for permission to reproduce this article.
There is a national trend towards the deployment of AR-15 rifles among law enforcement officers. For specialized units, short-barreled AR-15 rifles provide added maneuverability for indoor engagements such as breaching while serving warrants, responding to active shooters, or in urban settings. The trend towards the use of rifles for added accuracy and longer engagement distances comes with additional sound pressure levels associated with high-velocity projectiles. Relative to pistol cartridges, rifle ammunition is chambered at higher pressures and consequently produces significantly higher sound levels when measured at the same distances or with higher caliber rifles and supersonic ammunition. These levels are further increased when barrel length decreases.
The optimal strategy for measuring impulse noise, such as that produced by firearms, has been the topic of significant discussion. Brinkmann (2000) provides an excellent overview of the challenges, particularly the issue of impulse noise not falling squarely within acoustics, but rather under the realm of fluid dynamics, gas dynamics, and shock waves. Applied to rifles, this is due to the rapid expansion of gas into the atmosphere when the projectile leaves the muzzle which forms a spherical and often supersonic pressure wave. The chamber pressure inside an AR-15 barrel is rated in excess of 50,000 PSI and standard ammunition, depending on barrel length can have exit velocities as high as 3,200 feet per second.
In order to assess potential auditory risk, multiple essential parameters must be measured. These include peak overpressure, rise time, time-duration, impulse noise spectrum, and impulse noise energy (Brinkmann, 2000). Some of these properties are easier to measure than others, and the technical challenges have been well summarized by Rasmussen et al. (2009). Factors such as primer explosion and gas leak also influence the waveform, and may need to be considered as part of the overall sound source generation and propagation (Beck et al., 2011). Propagation does not occur equally in all directions, with forward propagation being much more significant than backwards propagation and right-left propagation being approximately equal, until the shooter’s head is factored in as a variable, at which time the head shadow effect reduces right or left ear sound pressure level measurements depending on shooter handedness and shooting style (Rasmussen et al., 2009).
One of the first considerations in measuring weapon discharge is the microphone. Rasmussen et al. (2009) carefully describe the effects of changing the direction of the microphone, from pointed toward the direction of the sound source (0 degree incidence), resulting in an increased sound pressure gradient in front of the microphone, to perpendicular to the direction of sound propagation (90 degree incidence), which allows sound to pass over the diaphragm but can modify the frequency input to the microphone. MIL-STD-1474D stipulates that transducers with a blunt cylinder shape “shall be positioned with the sensing surface facing up if possible,” (MIL-STD-1474D REQUIREMENT 4, 1997). The effect of incidence angle has been carefully explored by Moreland and Garinther (1965). However, relevant to this discussion, it is worth note that modern free-field microphones are designed to be pointed at a sound source, whereas pressure microphones are intended to be pointed perpendicular to the sound source. In field tests, we have used PCB electronics microphones of both types (free field: 378C01; pressure: 378A12), both of which have significant dynamic ranges appropriate for measurements of many (but not all) firearms. The impact of rotating either microphone by 90 degrees, into the “incorrect” orientation per the manufacturer guidance, has been approximately 2-dB difference in the measured peak sound pressure level.
In addition to careful microphone selection, pre-amplifiers, common to high intensity microphones, can and often do reduce the dynamic range over which microphones can respond (for review see Rasmussen et al., 2009). Thus, while best practices dictate a microphone that is capable of transducing signals up to 170 dB SPL for occupational noise and up to 195 dB SPL for weapon noise (Kardous et al., 2005), to the best of our knowledge, there are no commercially available condenser microphones that are capable of accurately transducing impulse sounds exceeding a maximum of approximately 180-184 dB SPL.
A second issue of fundamental importance is the selection of the recording device. It is not difficult to find examples of sound level meters being used to measure s firearm discharges (Branch, 2011; Guida et al., 2011). However, not only are the microphones unlikely to have adequate dynamic range to capture the extremely high level transients produced by a firearm discharge, but the sampling rate may also grossly underestimate the true sound pressure level. Most commercially available and professional sound level meters have a sampling rate on the order of 50 kHz (one sample every 20 microsec). This is significantly lower than the recommended 200 kHz sampling rate (one sample every 5 microsec) identified as best practice (Kardous et al., 2005). In field tests, we have used both a Larson-Davis model 831sound level meter set for impulse noise and a Pulse spectrum analyzer (3052-A-030, Brüel and Kjær) with a 256-kHz sampling rate. A comparison of both systems demonstrated approximately a 6-dB increase (or more) in the measured sound level when using the Pulse system relative to the sound level meter.
Over the past several months, we have been evaluating the sound pressure levels associated with varying AR-15 barrel lengths near the muzzle and at the level of the shooter’s ear; common locations in law enforcement for both the operator and other officers when deployed as a team (Le Prell et al., 2014). As part of an active collaboration between academia and local law enforcement, we have begun a systematic evaluation of the efficacy of suppressors (commonly called “silencers”) to determine the extent to which these devices can attenuate sound levels at a shooters ears, and parallel to the muzzle, positions where other members of the law enforcement team might be expected to be located. Our results, using a 256,000 sample/sec sampling rate, are showing significantly higher sound pressure levels at all unsuppressed barrel lengths relative to previous reports that used lower sampling rates. Sound pressure at the shooter’s ears decreased as weapon barrel length increased, a finding that is intuitively appealing. Specifically, as the barrel becomes longer, the point of discharge is further from the shooter ears, and sound levels are decreased. Conversely, as the barrel becomes shorter, the point of discharge is brought closer to the shooter, and sound levels are correspondingly higher. This is problematic, given increasing use of short-barreled AR-15 rifles for improved maneuverability by law enforcement. Particularly worrisome from a hearing conservation perspective, the higher sound pressure levels that we have measured may still underestimate the true sound pressure levels as certain rifle combinations produced sounds that exceeded the physical limits of condenser microphone technology, overloading the PCB 37812 microphone, with an upper dynamic range of 180 dB SPL.
It is beyond the scope of this brief report to detail our findings to date with suppressors. We do however point to one important observation. The peak sound levels produced by suppressed AR-15s, a weapon system that is commonly used by law enforcement personnel, have not been reduced to 140 dB SPL using any combination of barrel length and suppressor, at any location drawn from shooter ear and 1 meter left of the muzzle. However, it is important to note that suppression generally decreased unsuppressed peak levels by 5-20 dB, a finding in support of suppressor use. In Figure 1, we illustrate time waveforms for an unsuppressed AR-15 discharge, and a suppressed AR-15 discharge, for discharges produced from a more conventional platform, the 16” barrel length. Occupational Safety and Health Administration guidance states that, “Exposure to impulsive or impact noise should not exceed 140-dB peak sound pressure level,” (OSHA, 1983). Other organizations set this limit at 140-dB C-weighted SPL (American National Standards Institute, 1996) but these limits are largely considered outdated and incomplete (Kardous et al., 2005). New models have been proposed, such as the Auditory Hazard Assessment Algorithm for Humans (AHAAH) which is a computer-based model that was developed by Price and Kalb (Price & Kalb, 1991b; Price & Kalb, 1991a; Price, 2007), but there is an urgent need for additional data assessing the validity of this and other models used to predict the risk of hearing loss from a given noise insult (Smoorenburg, 1982; Smoorenburg, 2003). We agree with Starck et al. (2003), who have stressed the urgent need for a “single number” for noise risk assessment, although we note that with the known time-intensity trading relationship for occupational noise, it is unlikely that there will ever be a single number used to assess risk, as the total risk will always be a function of both time and level. In the case of impulse noise, this might be better extrapolated as number of impulses and level, but even that fails to capture the full relationship as the pattern of distribution of impulses over time clearly influences the hearing hazard (Danielson et al., 1991). Perhaps laboratory based studies assessing temporary threshold shift after lower level impulses will be helpful in providing some insight about the relative importance of different acoustic features within an impulse noise signal (following models such as those used by Spankovich et al., 2014), but any relationships established using small temporary threshold shift tests may not translate to more robust exposures that result in immediate permanent threshold shift, such as an unsuppressed, unprotected, unexpected weapon discharge. Given the current incomplete state of understanding of noise risk from firearm noise, and the incomplete reduction of sound levels by suppressors, earplugs and earmuffs must remain a first and foremost consideration for protection of human hearing during hazardous impulse noise exposure.
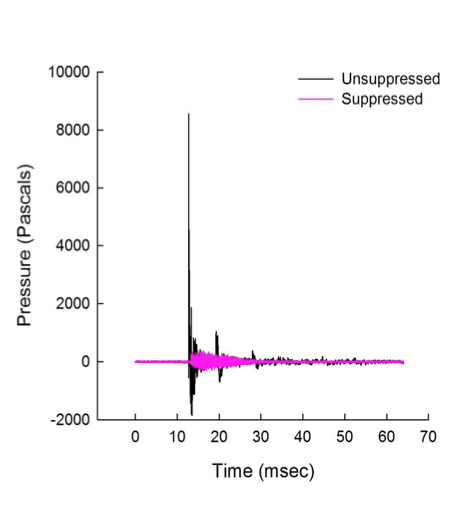
Figure 1. The time waveform for a single discharge from a 16” AR-15 is shown, with sound levels shown for both an unsuppressed and a suppressed condition. Levels were measured 1 meter left of the muzzle with the muzzle and microphone both fixed at a height of 5 feet 3 inches above the ground. Samples were acquired at a rate of 256,000 Hz using a Brüel & Kjær 3052-A-030 PULSE system in combination with Brüel & Kjær LabShop, Data Recorder, and Reflex software for waveform analysis. This specific waveform was acquired using a PCB piezoelectric pressure sensor (Model 113B27), as we have found that unsuppressed weapon discharge saturates recordings obtained using a PCB transducer microphone despite a manufacturer provided upper limit of 180-dB SPL (Model 378A12).
In summary, there is a significant acoustic overpressure danger associated with unsuppressed discharges of rifles and while “silencers” were effective at reducing peak SPL, these did not provide sufficient suppression for use without hearing protection. The widespread adoption of shorter-barrel rifles, which increases sound pressure levels at the shooter’s ears, carries increased risk factors for law enforcement officers related to long-term hearing health. Further experiments are needed to determine optimal barrel length/suppressor and weapon operating mechanism combinations for appropriate hearing conservation strategies.
Acknowledgements. The data in Figure 1 were collected in a study that used equipment purchased by the Hearing Research Center at the University of Florida, facilities and equipment from the High Springs Police Department and the Gainesville Target Range facility. The authors gratefully acknowledge the contributions of Ryan Scott and Christopher Spankovich to the data collection process. In addition, we are grateful for equipment loans and technical support from Brüel & Kjær, especially Jim Wyatt, Gert Nyrup, and Alfonso Moreira. We also thank David Butcher of the Alachua County Sheriff’s Office for volunteering his time, and Mark Ocepek and Steve Bottcher from the Gainesville Target Range for donating their time, facilities, and suppressors to this study.
Literature Cited
American National Standards Institute 1996. ANSI S3.44-1996, American National Standard Determination of Occupational Noise Exposure and Estimation of Noise-Induced Hearing Impairment. American National Standards Institute, New York.
Beck S.D., Nakasone H. & Marr K.W. 2011. Variations in recorded acoustic gunshot waveforms generated by small firearms. J. Acoust. Soc. Am., 129, 1748-1759.
Branch M.P. 2011. Comparison of muzzle suppression and ear-level hearing protection in firearm use. Otolaryngol. Head Neck Surg., 144, 950-953.
Brinkmann H. 2000. Techniques and procedures for the measuremente of impulse noise. Paper presented at the RTO HFM Lecture Series on "Damage Risk from Impulse Noise" held in Maryland, USA, 5-6 June 2000 and Meppen Germany 15-16 June 2000, and published in RTO-EN-11.
Danielson R., Henderson D., Gratton M.A., Bianchi L. & Salvi R. 1991. The importance of "temporal pattern" in traumatic impulse noise exposures. J. Acoust. Soc. Am., 90, 209-218.
Guida H.L., Diniz T.H. & Kinoshita S.K. 2011. Acoustic and psychoacoustic analysis of the noise produced by the police force firearms. Braz J Otorhinolaryngol, 77, 163-170.
Kardous C.A., Franks J.R. & Davis R.R. 2005. NIOSH/NHCA Best-practice workshop on impulsive noise. Noise Control Eng. J., 53, 53-60.
Le Prell C.G., Lobarinas E., Spankovich C. & Scott R. 2014. Quiet down. The effects of suppressors on short-barreled AR-15 rifles: Implications for Law Enforcement and the Military. 39th Annual National Hearing Conservation Conference- Stop Gambling with your Hearing; NHCA Spectrum, Volume 31, Supplement I, page 57.
MIL-STD-1474D REQUIREMENT 4 1997. Impulse noise in personnel occupied areas.
Moreland B. & Garinther G.R. 1965. Transducer Techniques for Measuring the Effect of Small-Arms' Noise on Hearing. U.S. Army Technical memorandum 11-65.
OSHA 1983. 29 CFR 1910.95. Occupational Noise Exposure; Hearing Conservation Amendment; Final Rule, effective 8 March 1983. U.S. Department of Labor, Occupational Safety & Health Administration.
Price G.R. 2007. Validation of the auditory hazard assessment algorithm for the human with impulse noise data. J. Acoust. Soc. Am., 122, 2786-2802.
Price G.R. & Kalb J.T. 1991a. Insights into hazard from intense impulses from a mathematical model of the ear. J. Acoust. Soc. Am., 90, 219-227.
Price G.R. & Kalb J.T. 1991b. A new approach to a damage risk criterion for weapons impulses. Scand. Audiol. Suppl., 34, 21-37.
Rasmussen P., Flamme G.A., Stewart M., Meinke D. & Lankford J. 2009. Measuring recreational firearm noise. Sound Vibrat., 43M, 14-18.
Smoorenburg G.F. 1982. Damage risk criteria for impulse noise. In: R.P. Hamernik, D. Henderson amp; R.J. Salvi (eds.) New Perspectives on Noise-Induced Hearing Loss. New York: Raven Press.
Smoorenburg G.F. 2003. Risk of hearing loss from exposure to impulse sounds. In: t.R.H.F.a.M.P.H. NATO Science and Technology Organization (ed.) RTO-TR-017 - Reconsideration of the Effects of Impulse Noise
Spankovich C., Griffiths S.K., Lobarinas E., Morgenstein K.E., de la Calle S., et al. 2014. Temporary threshold shift after impulse-noise during video game play: Laboratory data. Int. J. Audiol., 53 Suppl 2, S53-65.
Starck J., Toppila E. & Pyykko I. 2003. Impulse noise and risk criteria. Noise Health, 5, 63-73.
Photos courtesy of MCpl Patrick Blanchard, Canadian Forces Combat Camera